Electric vehicles are a nightmare for long distance drivers. Gas-powered vehicles can feasibly be driven from coast to coast (with a few stops for fuel, of course). But electric vehicles must stop more often for recharging to travel the same distance.
While some champion the green technology of electric vehicles, others complain about the inevitable “range anxiety” experienced by drivers. Electric vehicles that run on lithium-ion batteries typically have a range of around 100 miles per charge. On the other hand, gas-powered vehicles can run for 300 miles, three times the range of electric vehicles, for each stop at the gas station. So why not make electric vehicles with higher capacity to overcome this range issue?
Lithium-ion batteries are known for their high cyclability, which is a measure of the number of times they can be recharged before they begin to break down. The issue with these batteries, however, is their low energy density. Energy density is a metric of how much energy a battery can store for every unit of its weight. Because lithium-ion batteries use heavy transition metal components to operate, their energy density is low, and so is not optimized for bigger applications like electric vehicles. As a result, increasing the driving range for automotive applications by just adding more batteries would result in a significant weight gain and decreased overall performance.
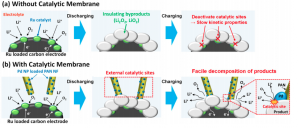
Of course lithium-ion batteries are not the only batteries around. Lithium-air batteries use a different set of chemical reactions devoid of transition metal components. They thus have a higher energy density. Unfortunately, lithium-air batteries have failed to gain commercial attention because of their low cyclability — they typically last only around 40 cycles before breaking down. Determined to overcome this barrier, a team of Yale researchers from the Transformative Materials and Devices Lab of Associate Professor of Chemical and Environmental Engineering André Taylor has recently published a paper in Nano Letters reporting a newly designed lithium-air battery architecture. The lead author of the paper is Yale postdoc Dr. Won-Hee Ryu, whose new development comes with both high energy density and cyclability. The product is already presenting exciting implications for sustainable energy storage and efficient energy consumption.
How it works
Lithium-air batteries operate by harnessing the power of electrochemical energy. At the heart of this energy are two oxidation-reduction reactions of lithium and oxygen. Oxidation-reduction reactions involve the movement of electrons between different atoms. In lithium-air batteries, this translates into the movement of electrons as current. When the battery delivers energy, or discharges, lithium ions react with oxygen to form different lithium oxides. In the charging phase, the battery uses an oxygen evolution reaction in which oxygen gas is given off as the lithium oxide products from discharging are decomposed back into lithium ions and oxygen gas.
These reactions are carried out in the presence of a catalyst because they occur too slowly at standard conditions. Chemical catalysts participate in reactions without being consumed. They are generally used to speed up important chemical processes. Catalysts can range in size from large enzymes that speed up digestion in the human body to small metal atoms that speed up recharging in a battery. In this new lithium-air battery architecture, a special porous polymer membrane is dotted with the metal catalyst palladium. The catalytic membrane is key to improving the cyclability of the new architecture.
Below the catalytic membrane is a large surface area electrode. A battery’s electrode is where electricity either enters or leaves. This electrode is dotted with another catalytic metal, ruthenium. Ruthenium was chosen in the Yale team’s design because it catalyzes both the oxydation-reduction reaction and the oxygen evolution reaction. Thus, its catalytic activity would benefit both the discharging and charging chemical reactions.
Circumventing potential problems
The lithium oxides produced during the discharging phase of the battery build up on the electrode and insulate it. Excessive buildup of insulating product, however, reduces the lithium-air battery’s cyclability by blocking the ruthenium reactions. Blocking such catalytic sites would have negative consequences for discharging and charging, quickly decreasing battery cyclability.
To resolve this issue, Yale researchers developed a mesoporous polyacrylonitrile (PAN) membrane. Mesoporous membranes have pores with a diameter between two and 50 nanometers. PAN itself is similar to a large chain of little plastic building blocks, which is essentially what comprises a polymer. This membrane was created through electrospinning — a technique that draws out thin fibers from the tip of a syringe of polymer solution. The tip is kept in an electric field, and when the field in the system is large enough, thin nanoparticles are uniformly distributed across the membrane. Additionally, the researchers were careful in making sure that particles were distributed such that they did not contact one another. This separation prevents the catalytic membrane from acting as a short circuit when in contact with the electrode.
Through delicate tweaking of the various components of the redesign, Ryu’s team created a lithium-air battery that avoided earlier problems. What next?
Power in the real world
Given that so much progress has been made on the lithium-air battery, why has it not yet been adopted as the standard for rechargeable batteries? Ryu explained that the current lithium-air battery architecture uses pure oxygen gas in place of air. Using pure oxygen increases the reaction rate for discharging. “Lithium ions tend to react with carbon dioxide in air, producing lithium carbonates that make the battery difficult to recharge,” Ryu said. Pure oxygen circumvents this issue. For practical purposes, however, lithium-air battery architecture requires the use of air instead of pure oxygen, since it would be impractical for devices such as cell phones and laptops to carry tanks of pure oxygen.
Still, what Ryu has found holds tremendous importance in the context of cell phones and laptops. Ryu explained that the higher energy density of the lithium-air battery will allow cell phones and laptops to have longer battery life in both the short-term and long-term. In the short-term, it will provide greater energy storage in the device. In the long-term, the greater cyclability of the battery will allow for more recharges. Not only would the new battery architecture affect small products, but it would have a similar effect on larger products.
Electric vehicles such as Tesla’s Model S would significantly benefit from a lithium-air battery system in several ways. Because of the higher energy density of lithium-air batteries, owners of electric vehicles would be able to overcome their range anxiety. “Tesla battery packs are the most expensive single component of the Model S,” Ryu said. If energy-dense lithium-air batteries replaced lithium-ion batteries, smaller battery packs could be used. Lowering the cost of electric vehicles’ most expensive component would allow these vehicles to become cheaper substitutes for gas-powered vehicles, opening up the possibility of a more convenient and efficient transportation system that runs on green.
Cover Image: Art by Casey McLaughlin.
About the Author: Sami Hakani is a freshman prospective chemistry major in Morse College. He is a writer for the Yale Scientific Magazine and is interested in organometallic chemistry research.
Acknowledgements: The author would like to thank Dr. Ryu for his time and enthusiasm for his research.