Ten thousand scientists and engineers from over one hundred countries. Over one thousand superconducting magnets, each more than fifty feet in length. Thirteen billion dollars. One thirty-eight-thousand-ton tunnel. This is what it took to build the Hadron Collider over the course of its ten-year construction. It was the largest and most powerful experimental facility built. The aim of the endeavor was to discover new particles, notably, the Higgs boson, by smashing protons together at speeds nearing the speed of light. But what if this same feat could be achieved on a tabletop using a gas chamber and a few lasers?
Researchers in Professor Dave DeMille’s group at Yale have improved a complex technique in atomic physics to explore this exciting physical topic. The technique, called laser cooling and trapping, combines atomic spectroscopy with the mechanics of light to change the properties of gaseous atoms and molecules. By shining lasers at gas molecules from several directions, researchers were able to cool the molecules to near absolute zero and levitate them in space, allowing for extremely precise measurements for a wide array of applications.
Cooling Atoms with Lasers
The concept of shining a laser at an object to cool it down is not intuitive, especially since we usually think of lasers as heat sources. Laser cancer treatment, 3D printing, and laser cutting all use the high energy of laser light. Using a laser as a cooling agent seems odd, since it is difficult to imagine using high energy laser light to decrease the energy of a group of atoms or molecules.
Temperature is a measure of the average velocities of atoms or molecules, so in essence, too cool something is to slow down its atoms and molecules. But to slow an atom down, the atom needs to be pushed in the opposite direction of its motion. This can be achieved on an atomic scale by making use of the properties of atoms and light. The photons of laser light carry momentum and energy, and when they hit an atom moving in the opposite direction, the momentum is transferred from the photon to the atom, slowing the atom down. When enough photons hit the atom, the atom could stop moving altogether.
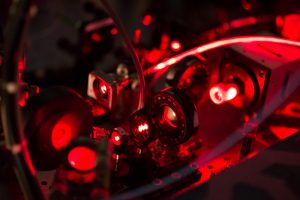
However, the photons in laser light only interact with an atom if they have a certain, extremely precise frequency. This is complicated by the fact that atoms are in constant motion. The trick of laser cooling is to deliberately adjust the frequency of laser light so the frequency is slightly smaller than the frequency that would be needed to excite an atom that is at rest. This creates what is called a Doppler shift, more commonly known to affect the way sound waves are perceived. For example, if an object emitting sound moves away from a listener, he or she will hear a lower pitch, whereas if the object moves toward the listener, he or she will hear a higher pitch. This principle holds true for light as well as sound. If an atom is moving toward a laser, the atom experiences a frequency slightly higher than the frequency of laser light, causing the atom to absorb the photon. If an atom is moving away from a laser, the atom experiences a frequency too low for photon absorption. “If the laser is calibrated such that it has a frequency too low to excite an atom at rest, but such that if that atom starts moving toward the laser, the Doppler shift makes the atom ‘see’ the correct frequency, then an electron transition can occur and the photon will be absorbed,” said DeMille.
Once enough photons from one laser hit an atom, the atom will slow down and eventually stop moving. Once stopped, the atom also ceases to absorb photons, because the atom only absorbs photons when moving toward the laser. For this process to work, several lasers are placed around the collection of atoms. Any given laser only slows down atoms moving toward that laser. But since atoms travel in all directions, each atom needs to be individually Doppler shifted using several lasers. The net result is that all of the atoms stop moving. Since the speed of an atom is directly related to its temperature, this technique can chill the atoms to less than a thousandth of a degree above absolute zero.
A Magneto-Optical Trap for Molecules
The process of cooling atoms with lasers is only useful if they can be simultaneously confined in space such that researchers can study their properties. To achieve this, researchers now use a technique in cold-atom physics called the magneto-optical trap (MOT), which combines laser cooling with restoring forces which compress a cloud of gaseous atoms into a tight ball. “The magneto-optical trap technique is so ubiquitous that pretty much anything you do in the field of atomic physics uses it. It is really that revolutionary. These systems are now the best controlled systems in the world,” said Matthew Steinecker, a fourth-year graduate student at Yale.
The magneto-optical trap has been used to simultaneously cool atoms down and levitate them in space for over three decades. David DeMille’s group, however, was the first group in the world to use the magneto-optical trap to cool and confine molecules. Molecules, unlike atoms, have more complex internal structures, which pose additional technical challenges when trying to confine them using magneto-optical trapping. Molecules have additional properties, notably vibration and rotation, which makes it challenging to apply the same technique for molecules as was previously used for atoms.
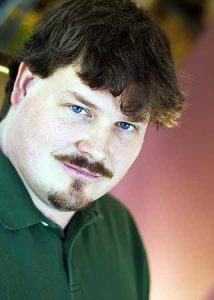
Image courtesy of David DeMille
Researchers in the DeMille group used a modified version of MOT to generate ultracold, trapped strontium monofluoride (SrF) molecules. The new technique, called radio-frequency magneto optical trap (RF-MOT), rapidly and simultaneously reverses the polarization of the lasers and the magnetic field of the system to counteract the challenges in controlling molecular movement when molecules vibrate and rotate. SrF molecules have less potent vibrational and rotational motions which make them easier to study. Refining this technique, researchers have been able to trap SrF molecules at a much greater density than has previously been achieved and at molecular temperatures as low as two-hundred fifty microkelvin, four thousand times colder than the Boomerang Nebula, the coldest naturally occurring place in the universe.
Beyond the Lab
The emergence of MOT technology has given rise to a number of exciting applications for ultracold matter. First, ultracold atoms and molecules allow for extremely precise measurements, making them ideal candidates for high-resolution spectroscopy, quantum measurements, and precision tests on the fundamental laws of nature. Ultracold atomic physics has already given rise to high-accuracy atomic clocks, which are the heart of satellite and GPS systems, quantum sensors, and precision measurements of well-known constants in nature such as the acceleration of gravity. We now know the value of the gravitational constant a million times more accurately using laser cooling and trapping than from previous experiments. MOT technology can detect and measure very small quantities of rare isotopes. Doctors can now measure the amounts of rare isotopes of calcium in the bones of patients with certain types of bone degeneration.
The Heisenberg-Uncertainty Principle states that the energy and time of a particle cannot be simultaneously measured with high precision. The longer amount of time an object can be observed, the better one can measure its energy. Using MOT technology which simultaneously confines molecules in space and cools them down, the energy of atoms, and now molecules, can be measured with extreme precision. The DeMille group has recently embarked on an experiment using RF-MOT to trap molecules and observe very subtle changes in energy of molecules that are caused by elemental particles, the building blocks of the universe, including the Higgs boson. This could even lead to the discovery of new elementary particles.
The complex and information-rich nature of molecules is opening the door to a number of exciting new experiments using laser cooling and trapping technology. The unique properties of molecules enhance researchers’ ability to test our understanding of fundamental physics. Researchers are currently hunting for the permanent electric dipole moment of the electron. Since molecules have electric dipoles, they interact strongly through electric fields even when they are far apart. DeMille suggests that when a group of molecules is cooled to low enough temperatures, it will form states of matter with properties not-yet observed. “There’s a lot of interest in studying these exotic phases of matter where the pieces of the gas interact with each other pretty strongly even when they are far apart,” said DeMille.
Advancements in RF-MOT technology in the DeMille group are opening the door to a new set of experiments that will potentially revolutionize our understanding of fundamental physics. “There are certain observations that we can make about the universe that we don’t understand why it should be this way given the laws of physics. This is one area where we can look to see how our understanding of the laws of physics are subtly wrong,” said Steinecker. Laser cooling and trapping technology is on the verge of making a truly extraordinary catch.
Featured Art by Catherine Yang
About the Author:
William is a freshman Biophysics & Biochemistry major in Morse College. He is the copy editor for the Yale Scientific Magazine and works in Professor Forscher’s lab studying cytoskeletal dynamics underlying growth cone motility in neurons.
Acknowledgements:
The author would like to thank Professor DeMille and Matthew Steinecker for their passion and dedication to their research.