
Einstein’s theory of relativity may imply that we will never observe an object moving faster than the speed of light, but that has not stopped scientists from moving particles at speeds close to it. Particle accelerators allow scientists to give small-massed particles, such as protons and electrons, a substantial amount of kinetic energy to collide with targets in order to study subatomic particles.
Though there are differing theories on the nature of subatomic particles, the Standard Model is a generally accepted theory of what matter is and what holds it together. This model suggests that everything is made up of particles called quarks, leptons, and force carrier particles.
Particle Accelerators 101
For the past eighty years, scientists have used the particle accelerator to find out more about these units of matter. The basic mechanism of the accelerator has not changed over its history. A charged particle travels through an electric potential difference, using the energy of the electric field to gain energy and travel at increasing speeds. Thus, the particle is accelerated. Particle accelerators are tools not only for studying matter at its fundamental level, but are also used in X-ray generators and cathode ray tube (CRT) television sets. However, in these “low-energy” machines, a simple setup of a pair of electrodes and a direct current (DC) voltage is used.
In contrast, linear “high-energy” accelerators use a series of plates called “drift tubes” and an alternating current (AC) voltage. This allows the particles to accelerate through each drift tube and align with the sinusoidal voltage to reach high speeds.
The most widely used particle accelerators fall into two categories: linear and circular. As the name implies, a linear particle accelerator allows a particle to travel in a vacuum down a long, straight tube until it collides with a target.
Circular accelerators work via a similar principle, but instead use electromagnets; they have the primary advantage of creating continuous acceleration. Thus, circular accelerators tend to be smaller in size than linear accelerators of comparable power.
One key disadvantage, however, is their emission of a type of radiation called synchrotron radiation. This radiation creates some energy loss as “synchrotron light,” and thus the energy must be restored by the accelerating cavities (analogous to the differing voltage plates in a linear accelerator) to maintain the high energy beam. The effect is magnified as the particles in the beam approach the speed of light.

Linear accelerators, or “linacs,” have a variety of uses in physics. Currently, the longest linac in the world is housed at Stanford University and spans approximately two miles. Through this tube, electron-positron collisions are observed in order to study the differences between matter and antimatter through the emission of particles called B mesons.
Using high energy beams – a 3 GeV positron beam and a 9.1 GeV electron beam – collisions between positrons and electrons can be observed to produce Y(4S) mesons, which then decay into equal numbers of B and anti-B mesons. From this, physicists at Stanford hope to explain the absence of anti-particles in everyday existence.
While similar projects are being conducted at other facilities across the globe, the Stanford Linear Accelerator Center (SLAC) is unique in its capabilities to research areas in particle physics and astrophysics, due partially to its incredible length and its ability to emit extremely high energy positron and electron beams.
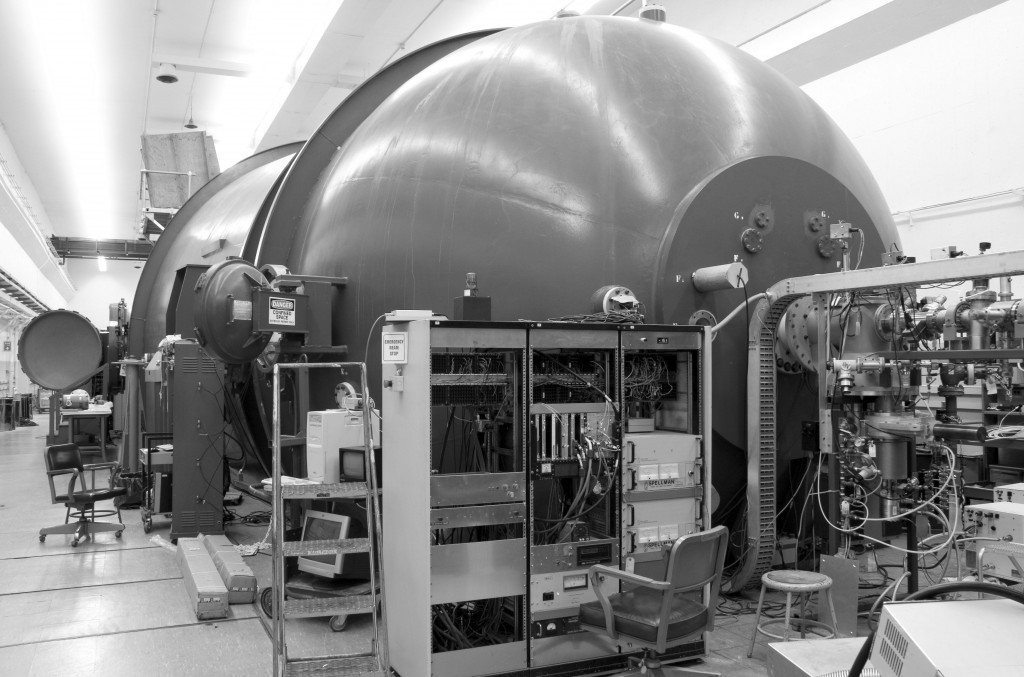
CERN: An International Collaboration
The European Organization for Nuclear Research, known as CERN, contains perhaps a more impressive set of accelerators for use by particle physicists. CERN is financially supported by twenty countries in Europe, and nearly 8000 engineers representing about 580 universities and 80 nationalities collaborate yearly on projects such as the Large Hadron Collider (LHC).
CERN operates a system of six different particle accelerators and one decelerator (which operates under the same principle as an accelerator, except for a reversed potential difference to induce deceleration). The system includes several linear accelerators and the circular accelerators 28 GeV Proton Synchrotron (PS) and 450 GeV Super Proton Synchrotron (SPS).
The SPS, with a diameter of approximately two kilometers, can be operated as a proton-antiproton collider and as a mechanism for injecting electrons, positrons, protons, and heavy ions into other colliders, including the Large Hadron Collider (LHC) and the Large Electron-Positron Collider (LEP), decommissioned in the year 2000.
As of May 2008, the activities at CERN have been primarily devoted to the formulation and construction of experiments related to the LHC. Unfortunately, by Fall 2008, it was clear that the LHC would not be operational until 2009.
Two major experiments, called ATLAS and CMS, intend to analyze the particles produced by collisions in the LHC and are “designed to investigate the largest range of physics possible.”

The next two sets of experiments, ALICE and LHCb, are described to be of “medium-size” and will address more specific issues with specialized detectors. For example, ALICE — “A Large Ion Collider Experiment” — will attempt to recreate the conditions just after the Big Bang. Lastly, two smaller sets of experiments, TOTEM and LHCf, will focus on “forward particles”, or heavy ions and protons.
Particle accelerators play key roles in diverse fields, including radiosurgery and radiotherapy, material analysis, isotopic dating, and ion implantation. Though the physics behind them can get quite complicated, particle accelerators offer an exciting look into matter’s composition.
Physicists hope that experiments like the six at CERN and others soon to come will continue to delve deeper into the nature of the fundamental units of matter.
Acknowledgements
The author would like to thank Dr. Stephen Irons for sharing his insights on particle accelerators used at Yale for the purposes of this article.
Further Reading
- Amaldi, Ugo. “The Importance of Particle Accelerators” Europhysics News. 2000. European Physical Society. https://www.europhysicsnews.com/full/06/article1/article1. html
- “The Elegant Universe.” NOVA. 2006. PBS.com. https://www.pbs.org/wgbh/nova/elegant/
- European Organization for Nuclear Research. 2007. CERN. https://public.web.cern.ch/Public/
- “SLAC Public Websites.” Stanford Linear Accelerator Center. 2008. Stanford University. https://www-public.slac.stanford.edu/
- “Particle Accelerator Theory.” SLAC Virtual Visitor Center. 2008. Stanford University. https://www2.slac.stanford.edu/vvc/theory/