Photography by Paul-Alexander Lejas
As energy demands soar and environmental concerns grow, the search for more efficient technologies has never been more urgent. Superconductors—materials that can conduct electricity with zero resistance—offer an enticing solution. They allow for continuous flow of energy without loss. But there’s a catch: so far, superconductors only work at extremely low temperatures. Understanding the underlying mechanisms behind superconductivity is essential to improving their performance and making them viable for widespread use.
A research group at Yale, led by assistant professor of physics Eduardo H. da Silva Neto, has uncovered a key piece of the puzzle. Their new study, published in Nature Physics, provides experimental evidence that nematicity—an electronic phase that breaks the rotational symmetry of electrons—plays a crucial role in enabling superconductivity in certain iron-based materials. “There’s a wide range of [iron-based] materials which can superconduct […] and that have a nematic phase,” said Pranab Kumar Nag, a postdoctoral researcher in the da Silva Neto Lab and lead author of the paper. Traditional models cannot fully account for how superconductivity occurs in some of these materials. Theorists have long suspected that fluctuations associated with the nematic phase could drive superconductivity. Yet, concrete proof of this mechanism remained elusive until now.
“This is the first time, as far as we know, that nematic fluctuations have been found as evidence for the driver of superconductivity,” said Kirsty Scott GSAS ’26, a co-author on the paper. By helping us understand the driving mechanisms behind superconductivity, this research brings us one step closer to achieving the “holy grail” of materials science: a superconductor that works at room temperature.
Electrons That Attract
At its core, electricity is simply the movement of electrons. As these negatively charged particles flow through a material, they transfer energy, powering everything from light bulbs to supercomputers. But there’s a problem: electrons naturally repel each other. As they move, the constant jostling and bumping leads to energy loss in the form of heat. This loss is called electrical resistance. The amount of resistance depends on the material—metals like copper are excellent conductors with relatively low resistance, while rubber, with its high resistance, acts as an insulator.
Superconductivity flips this entire concept on its head. Instead of repelling each other, electrons in a superconductor form couples called Cooper pairs that attract each other and move effortlessly through the material with zero resistance. “To create this attraction, you need something to change the energy landscape of your electrons and allow them to now be attracted to each other,” Scott said.
But what allows for the creation of Cooper pairs? The answer for classical superconductors, discovered in 1911, lies in lattice vibrations—tiny oscillations of atoms within the material. As an electron moves, it distorts these vibrations, creating a wave that pulls a second electron along with it. This pairing mechanism allows electrons to glide together through the material unimpeded. However, the mechanism only works at ultra-low temperatures, close to absolute zero. At higher temperatures, the atoms oscillate more rapidly, disrupting the Cooper pairs and breaking the material’s superconductivity.
In the 1980s, scientists discovered a new class of superconductors, known as unconventional superconductors, that function at much higher temperatures. Unlike their classical counterparts, these materials don’t rely on lattice vibrations to form Cooper pairs, but the exact mechanism behind their superconductivity is still unclear and may differ between materials. Investigating this mechanism is a key focus of the da Silva Neto Lab.
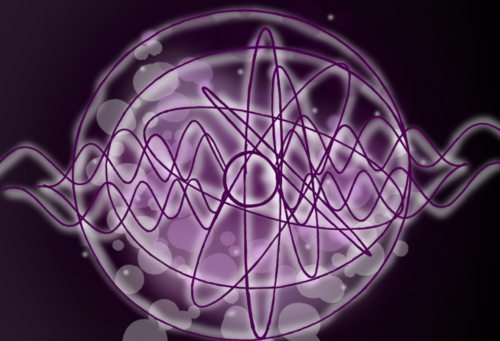
A Sea of Spin
So how do these unconventional materials form Cooper pairs at such high temperatures? The key is understanding the “phases,” or states, that electrons can inhabit. Just like how compounds can exist in three different states of matter—solid, liquid, or gas—electrons in a material can also arrange themselves into distinct quantum phases. Unlike conventional phases, which are determined by temperature and pressure, quantum phases arise from the collective behavior of electrons and their interactions. Observing and studying these phases requires extremely low temperatures. At higher temperatures, electrons and atoms gain energy and move more freely, making it difficult to distinguish the underlying quantum effects. Lowering the temperature slows down the motion, allowing quantum phases to emerge more clearly and enabling precise measurements of their properties.
One key example is the magnetic phase. Electrons, being charged particles, generate tiny magnetic fields due to an intrinsic property called spin. In most materials, electron spins point in random directions, canceling out any large-scale magnetism. However, under certain conditions, the spins can align in an orderly pattern, forming a magnetic phase. For example, in a ferromagnet like iron, all the spins point in the same direction, creating a strong magnetic field. In an antiferromagnetic phase, neighboring spins alternate directions, canceling out the net magnetization but still maintaining an ordered magnetic structure.
But electron spins aren’t static; they fluctuate and interact with each other constantly. This dynamic behavior is known as spin fluctuations. A helpful way to understand this is through phase transitions, like ice melting into water. While to the human eye ice becomes liquid at a well-defined temperature, on a microscopic level, regions within the ice can behave like a liquid even before the entire structure has melted. Similarly, even outside a true magnetic phase, spin fluctuations create local, short-lived regions where magnetism begins to emerge.
In unconventional superconductors, these fluctuations are extremely important. It is commonly believed that in many such materials, spin fluctuations can act as the glue that binds pairs of electrons together. Imagine a sea of electrons with their spins constantly fluctuating. As the temperature decreases, neighboring electrons can “feel” each other’s fluctuations. This creates an effective attraction between electrons with opposite spins, forming Cooper pairs.
Breaking Symmetry
Another type of quantum phase is the nematic phase, or nematicity. In this phase, electrons break rotational symmetry while preserving translational symmetry. What’s the difference? A circle, for example, has rotational symmetry because it looks the same from any angle. Meanwhile, a checkerboard has translational symmetry, since shifting it by a certain distance does not change its pattern. In a nematic phase, electrons are arranged so that translational symmetry remains intact, but their behavior is no longer the same in all directions. Like a crowd looking towards the stage at a concert, the electrons all orient in the same direction. This tendency for electrons to favor a specific direction in nematic phases is termed “anisotropy.”
Nematicity has drawn much attention in iron-based and high-temperature superconductors. In these systems, electronic nematicity emerges when the electronic structure exhibits anisotropy, despite the underlying atomic structure of the material remaining nearly symmetric. This suggests that nematicity is driven by electron interactions rather than by distortions in the atomic arrangement—in other words, it’s only the electrons at work. Similar to spin fluctuations, nematic fluctuations can create temporary, localized areas of nematicity that break rotational symmetry but are neither stable nor intrinsic to the material.
But does nematicity actually lead to superconductivity? This is a hard question—nematicity in iron-based superconductors was observed for the first time less than a decade ago, and researchers have faced major obstacles in their efforts to investigate further. One major challenge is that the nematic and magnetic phases in these materials occur under nearly the same thermodynamic conditions, making it difficult to determine whether they are interdependent or if nematicity can even exist without magnetism. In theory, nematic fluctuations could serve the same function as spin fluctuations, allowing electrons to form Cooper pairs by altering how they interact with one another. While superconductivity mediated by nematic fluctuations is well-supported in theory, experimental confirmation has been elusive due to this overlap.
Experimental Evidence
To investigate nematicity in superconductors, the research team focused on one of the few known families of materials where a true nematic phase does not overlap with a magnetic phase—sulfur-substituted iron selenium. These materials are characterized by a basic structure of alternating iron and selenium atoms, with selenium occasionally replaced by sulfur. To probe the electronic structure of these materials, the team used scanning tunneling microscopy and spectroscopy, a powerful technique capable of imaging sample surfaces and their electronic properties at the atomic level. “In our lab, we have a very sophisticated experimental setup. We do all of the experiments at a very low temperature, […] close to zero Kelvin. At that temperature, you can see all the quantum effects,” Nag explained. Each measurement could take several days to a week and required a highly controlled, noise-free environment to ensure high-quality data. Careful analysis was essential, and the group collaborated with international research teams to strengthen their conclusions for further verification.
The team’s findings show that at a certain substitution threshold, nematic fluctuations played a key role in driving superconductivity. However, localized spin fluctuations are still present and cannot be ruled out entirely. “We found that nematic fluctuations have at least taken over as the more prominent driver, but [we can’t] go as far as to say that spin fluctuations aren’t still important,” Scott explained.
Looking ahead, the da Silva Neto group plans to continue studying this family of iron-based materials, as well as other unconventional superconductors. Their work provides a fresh perspective on the potential drivers of superconductivity, bringing us closer to a more comprehensive understanding of unconventional superconductors. This knowledge could eventually enable scientists to harness superconductivity for breakthroughs that could revolutionize energy grids, computing, and transportation.