In the past few decades, scientists have increasingly delved into the field of synthetic biology. As its name suggests, synthetic biology combines the realms of biology and engineering to produce systems never before seen in nature. Often, these systems are inspired by what is found in nature, but they proceed a step further by undergoing “engineering” to become something more beneficial. In the context of synthetic biology, engineering typically refers to the introduction of foreign genetic material with “instructions” that tell the organism of interest what to produce. However, synthetic biology is not limited to simply expressing a biological product. In fact, scientists have come up with a number of ways to engineer organisms to do unexpected, beneficial things. For example, have you ever considered designing bacteria that act as pressure sensors?
While such a physical device made up of microorganisms seems difficult to imagine, researchers at Duke University have accomplished just that. By engineering self-patterning bacteria that can be printed on permeable, three-dimensional scaffolds, they generated pressure sensors out of microbes. The sensors are dome-shaped and are made of both organic and inorganic materials, namely gold nanoparticles applied onto the bacteria.
In a study published in early October, the researchers engineered a specific strain of Escherichia coli (E. coli), a bacterium commonly used in biological research, to produce a protein that composes the pressure sensor. “The main motivation is to demonstrate the following principle: living cells can be engineered to form self-organized 2D or 3D structures, which in turn can be used to assemble structured materials with well-defined physical properties,” said Lingchong You, the head researcher on the study and a biomedical engineering professor at Duke University.
The team chose to develop pressure sensors based on previous work at Harvard and MIT, where bacteria were engineered and applied onto pre-patterned, 2D surfaces to make electrically conductive biofilm switches that were controlled by an external electrode and supplemented with inorganic nanoparticles for conductivity. “Pressure sensing happens to be a function we used to demonstrate the idea above,” You said. “Our work represents a fundamentally new strategy to assemble structured materials with well-defined physical properties.”
To engineer a genetic circuit in E. coli that produced the pressure sensor’s scaffolding material, the researchers introduced foreign plasmids into the bacteria. Plasmids are circular pieces of DNA that carry a set of instructions telling the bacteria what to do. In this experiment, the foreign plasmids instructed the bacteria to produce a protein called curli, which acts as the building block to assemble the pressure sensor’s dome-like structure.
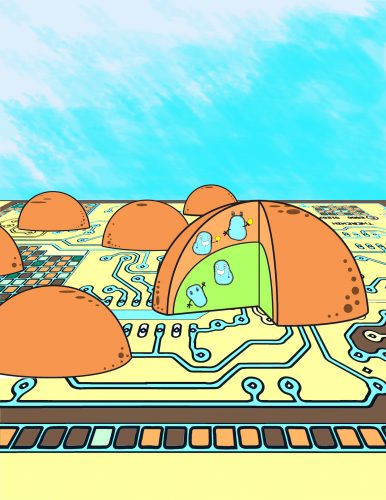
Following plasmid introduction, the researchers laid out a scaffolding design with a permeable membrane template—outlined using a modified inkjet printer—underneath growth media. The membrane provided structural support for both bacterial growth and the later addition of gold nanoparticles. After constructing the membrane support, a liquid culture of the bacteria was applied over the membrane. Individual bacterial colonies grew into the dome-like shapes, which researchers could control by adjusting the pore size and hydrophobicity (the extent of water-repulsion) of the membrane.
To complete the pressure sensor, gold nanoparticles were overlaid onto the bacterial colony domes after the colonies were fixed into place. The researchers hypothesized that the viscoelasticity—or resistance to shear stress and distortion—of the organic curli matrix, combined with the conductivity of the gold nanoparticles, could contribute to a functional organic-inorganic hybrid pressure sensor.
Indeed, this is what they observed. When two bacterial domes were moved to face each other and a constant electrical voltage was applied to the edge of a dome, the contact between both domes allowed electrical current to flow. One way to visualize this is by imagining that each bacterial dome is someone’s face. Just like the spark of a first kiss, when the two bacterial domes make contact, an electrical current is produced. Importantly, the strength of the current reflected the strength of the externally applied pressure. After further testing this relationship, both verifying and modeling the association, the researchers established that the bacterial domes could act as robust pressure sensors.
The investigation is a key step toward improving our understanding of how to program spatial patterns in cell populations, a topic within synthetic biology that has often been neglected. While this neglect is partially due to the difficulty of modeling spatiotemporal patterns over just temporal ones, other concerns include the difficulty of demonstrating such dynamics experimentally. In this investigation, the researchers not only addressed such concerns but also took a step beyond previous work by introducing the programming of self-organization. Here, the engineered bacteria were able to grow into their desired structure without any pre-patterning.
What do these bacterial pressure sensors hold for the future? “There are many opportunities and we’re currently pursuing some of these. We can imagine the generation of hybrid materials with other properties that can be used for diverse applications, including environmental cleanup and medicine,” You said.
Additionally, the researchers discussed the possibility of using curli to form other structures with different inorganic materials introduced. For example, if the gold nanoparticles were replaced with catalytic metal nanoparticles, catalytic structures could be built for many chemical and physical applications. Likewise, the curli protein itself could be replaced by other organic molecules to produce materials such as hydrogels. There is also the possibility of using other organisms, such as yeast, to create different pattern formations.
The future of this technology holds much promise. “I expect that the research in this direction will need to simultaneously address two related issues. One is to push the limit in terms of the diversity of materials that can be generated by living cells. The other is to generate specific types of materials for specific applications,” You added. Moving forward, their team hopes to expand on this work. “As the next step, we’re focusing on two directions: the generation of different kinds of spatial patterns, which remains a fundamental challenge in synthetic biology, and the implementation of different types of hybrid living materials,” You said.
Here at Yale, researchers at the Yale Microbial Sciences Institute are also employing synthetic biology to make new materials. One such scientist is Nikhil Malvankar, an assistant professor in the Yale Department of Molecular Biophysics & Biochemistry. Malvankar’s laboratory focuses on engineering soil bacteria that produce pili, a naturally conductive, filamentous protein that functions similarly to copper wires. His group aims to uncover the mechanisms of electron movement within these filaments, which act as nanoscale “wires” with tunable conductivity, to better understand how this system works at the molecular level and then to apply this knowledge to improve other bacterial systems. “The long term goals are to use synthetic biology for designing biomaterials and bioelectronics devices that will complement and extend current semiconducting technology,” Malvankar said.
Regarding the growing potential of synthetic biology, Malvankar highlights critical points to consider when working with microorganisms. “Bacteria are very adaptable organisms and employ multiple components and redundant pathways for cellular processes. Furthermore, bacteria can only function in limited environmental conditions such as physiological pH and aqueous environment,” Malvankar said. He also provided ideas for improving the bacterial pressure sensors. “In the future, it should be feasible to use living cells rather than fixed cells and also avoid expensive and toxic gold nanoparticles and use all-organic, biological systems.”
With all of these applications and more, the future of synthetic biology is very exciting. “In the current state of synthetic biology and bioengineering, one fundamental question is what things we can actually fabricate using living things. I hope to see different examples emerging from the community, which can further stimulate our imagination,” You said.